In a world where natural disasters are becoming increasingly frequent and devastating, the importance of earthquake-resistant building design cannot be overstated. These resilient structures not only save lives but also protect valuable assets. This article link into the intricate world of earthquake-resistant building design, exploring the key principles, innovative technologies, and the future of seismic architecture.
Earthquakes are natural that have shaped our planet for millions of years. However, in today’s urbanized world, these geological events pose a significant threat to human lives and property. To mitigate this risk, architects and engineers have been developing innovative earthquake-resistant building design solutions.
Understanding Earthquakes
Before delving into design solutions, it’s essential to grasp the science behind earthquakes. These are caused by the sudden release of energy in the Earth’s crust, resulting in seismic waves that can cause structural damage. Understanding the mechanics of earthquakes is the first step in designing resilient buildings.
Earthquake-prone regions are categorized into seismic zones based on historical seismic activity. Engineers conduct thorough risk assessments to determine the level of earthquake resistance required for structures in these zones. This assessment informs design decisions.
Throughout history, we’ve witnessed the catastrophic consequences of earthquakes on poorly designed structures. Examining past failures and successes in earthquake-resistant building design provides valuable insights into the evolution of this field.
Earthquake Resistance Design Standards Worldwide
Earthquake resistance design standards are crucial in ensuring the safety and resilience of buildings and infrastructure in regions prone to seismic activity. These standards vary from country to country, reflecting local geological conditions, historical seismic events, and engineering expertise. Here are some noteworthy earthquake resistance design standards from around the world:
1. United States – Building Code Standards
The United States has comprehensive seismic design standards established by the International Building Code (IBC) and the American Society of Civil Engineers (ASCE). These standards vary by region, with higher seismic design requirements in areas with a history of significant seismic activity, such as California. The seismic design standards in the U.S. include provisions for building materials, foundation design, and structural systems to withstand earthquakes.
2. Japan – Japanese Industrial Standards (JIS)
Japan is one of the most seismically active regions globally, and its earthquake resistance design standards are among the most stringent. Japanese Industrial Standards (JIS) govern various aspects of earthquake-resistant construction, including building materials, foundation design, and seismic retrofitting. Japan’s seismic design standards are continuously updated based on the latest seismic research.
3. New Zealand – New Zealand Building Code
New Zealand, located on the Pacific Ring of Fire, has developed a robust seismic design standard as part of its Building Code. The code includes provisions for earthquake-resistant design, with an emphasis on ensuring both structural integrity and occupant safety. New Zealand’s seismic design standards have evolved significantly following major earthquakes, such as the Christchurch earthquakes in 2010 and 2011.
4. Chile – NCh433 Building Code
Chile, another seismically active country, has established earthquake resistance design standards in its NCh433 Building Code. These standards consider factors like soil conditions, seismic hazard levels, and building types. Chile’s codes have been refined over the years to enhance the earthquake resistance of structures.
5. Italy – Eurocode 8
Italy, situated in a region with moderate to high seismic activity, follows Eurocode 8 for seismic design standards. Eurocode 8 provides guidelines for seismic-resistant design and retrofitting of buildings and infrastructure throughout Europe. Italy’s seismic standards are crucial for preserving historical structures while ensuring their earthquake resilience.
6. India – IS 1893 Building Code
India has its earthquake resistance design standard, IS 1893, which outlines seismic zoning maps and specifications for construction in various seismic zones across the country. Due to India’s vast geographical expanse, the standards consider diverse geological conditions and earthquake risks.
7. China – GB50011 Building Code
China’s seismic design standards are regulated by the GB50011 Building Code. These standards are crucial in a country with a wide range of seismic risks. China’s approach includes seismic-resistant design, construction materials, and retrofitting measures to enhance the resilience of buildings and infrastructure.
8. Greece – Greek Seismic Code
Greece, located in a seismically active region, has its Greek Seismic Code. This code addresses earthquake resistance in construction and is essential for preserving both ancient and modern structures in Greece.
In conclusion, earthquake resistance design standards are essential for mitigating the impact of seismic events on buildings and infrastructure. These standards are tailored to specific geological and seismic conditions in each country, reflecting years of research, lessons learned from past earthquakes, and a commitment to safeguarding lives and property. As seismic science and engineering continue to advance, these standards will evolve to meet the ever-changing challenges posed by earthquakes around the world.
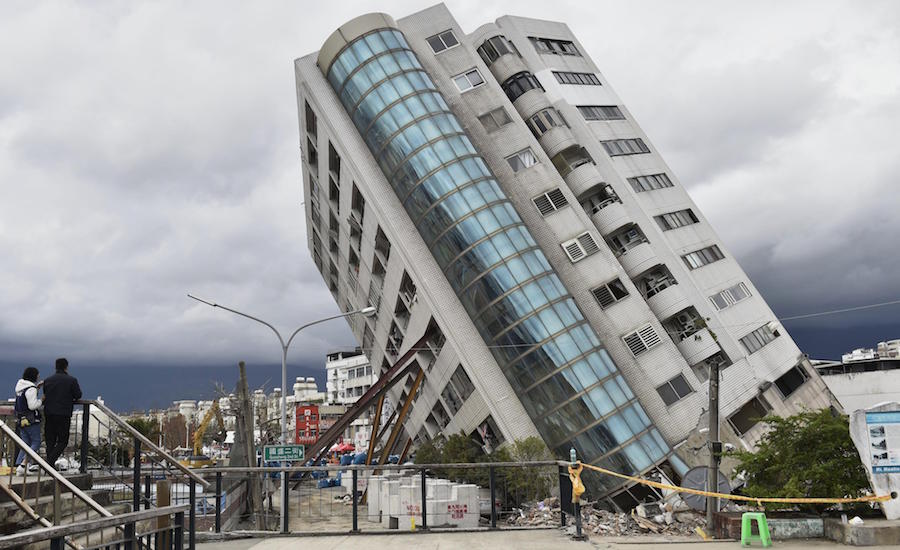
Seismic Load Evaluation: Ensuring Structural Resilience
Seismic load evaluation is a fundamental step in earthquake-resistant building design. It involves assessing the dynamic forces and ground motions that a structure may experience during an earthquake. Understanding these forces is essential for designing buildings and infrastructure capable of withstanding seismic events. Here are some key concepts related to seismic load evaluation:
1. Seismic Hazard Assessment
Seismic hazard assessment involves determining the level of earthquake risk in a particular region. This assessment considers factors such as historical earthquake data, geological conditions, fault lines, and ground motion predictions. It results in the creation of seismic hazard maps, which classify areas into different seismic zones based on the likelihood and intensity of earthquakes.
2. Seismic Design Ground Motion
Once the seismic hazard is assessed, engineers calculate the expected ground motion at a specific location. Ground motion is characterized by parameters such as peak ground acceleration (PGA), spectral acceleration (Sa), and ground motion response spectra. These values provide critical data for seismic load evaluation.
3. Response Spectrum Analysis
Response spectrum analysis is a method used to evaluate how a structure will respond to ground motion at different frequencies. It involves creating a response spectrum curve that represents the building’s response at various vibration frequencies. Engineers use this analysis to design structures with natural frequencies that align with the seismic forces expected in the area.
4. Equivalent Static Analysis
Equivalent static analysis simplifies the dynamic earthquake forces into a static equivalent, making it easier to apply in structural design. It involves estimating the peak ground motion and then applying it as a uniform lateral force on the structure. While this method simplifies calculations, it may not capture the full dynamic behavior of the building.
5. Dynamic Analysis
Dynamic analysis is a more advanced approach that considers the complex behavior of a building during an earthquake. It takes into account factors such as building stiffness, mass distribution, and damping. There are two primary methods of dynamic analysis: time history analysis, which uses recorded earthquake data, and response spectrum analysis, which uses predefined ground motion spectra.
6. Seismic Load Combinations
Seismic load evaluation includes considering various load combinations, including seismic loads, gravity loads, and other environmental factors. These combinations ensure that the structure is designed to withstand not only seismic events but also everyday loads.
In seismic design, structural engineer needs to consider various load combinations to ensure that buildings and structures can withstand the forces generated during an earthquake. These load combinations are typically prescribed by building codes and seismic design standards and are used to assess the structural integrity and safety of a building. The specific load combinations may vary by region and building code, but here are some common load combinations for seismic design:
- Dead Load (DL) + Seismic Load (EL): This combination considers the permanent weight of the structure and the seismic forces acting on it. The seismic load is usually determined using response spectrum analysis or equivalent lateral force procedures.
- Dead Load (DL) + Live Load (LL) + Seismic Load (EL): This combination includes the effects of both the permanent and live loads, along with the seismic forces. Live loads are temporary loads caused by occupants, furniture, equipment, and other variable factors.
- Dead Load (DL) + Snow Load (SL) + Seismic Load (EL): In areas prone to heavy snowfall, this combination accounts for the weight of snow, along with the dead load and seismic forces.
- Dead Load (DL) + Wind Load (WL) + Seismic Load (EL): For regions with high wind loads, this combination considers the effect of wind forces in addition to dead load and seismic forces.
- Dead Load (DL) + Snow Load (SL) + Live Load (LL) + Seismic Load (EL): This combination takes into account the combined effects of dead load, snow load, live load, and seismic forces, which is relevant in regions with cold climates and the possibility of seismic activity.
- Dead Load (DL) + Wind Load (WL) + Live Load (LL) + Seismic Load (EL): This load combination is used in areas susceptible to both strong winds and seismic activity. It considers the simultaneous effects of these loads along with live and dead loads.
- Dead Load (DL) + Roof Live Load (LLr) + Snow Load (SL) + Seismic Load (EL): In regions with sloped roofs, this combination accounts for the roof’s live load, snow load, and seismic forces in addition to the dead load.
- Dead Load (DL) + Wind Load (WL) + Seismic Load (EL) + Foundation Uplift: This combination includes the effects of wind, seismic forces, and potential uplift forces on the foundation, which may occur in areas with expansive soils or high groundwater levels.
It’s important to note that the specific load combinations and their factors may vary based on local building codes and the characteristics of the structure. Engineers must carefully analyze and design structures to ensure their safety and compliance with applicable regulations, including seismic design codes.
7. Seismic Load-Resisting Systems
Structural engineers use various seismic load-resisting systems, such as shear walls, moment frames, braced frames, and base isolators, to dissipate and distribute seismic forces. The choice of system depends on the building’s design, size, and expected seismic loads.
8. Performance-Based Design
Performance-based design is an innovative approach that focuses on the desired performance of a building during an earthquake. Instead of solely meeting code-prescribed minimums, engineers establish specific performance objectives, such as preventing structural collapse or ensuring occupant safety.
9. Seismic Retrofitting
Seismic load evaluation is also crucial for assessing existing structures and implementing retrofitting measures to enhance their earthquake resilience. Engineers evaluate the structure’s capacity to withstand current seismic loads and recommend retrofitting solutions as needed.
Seismic load evaluation is a multifaceted process that requires a deep understanding of earthquake dynamics and structural behavior.
It is the foundation upon which earthquake-resistant building design is built, ensuring that structures can withstand the forces of nature and protect lives and property during seismic events.
As seismic science and engineering continue to advance, seismic load evaluation techniques will evolve to enhance the safety and resilience of our built environment.
Principles of Earthquake-Resistant Design
Strong Foundations
A strong foundation is crucial in withstanding seismic forces. Deep pile foundations and innovative base isolators can enhance a building’s stability during an earthquake.
Flexible Structures
Buildings designed to flex and sway during an earthquake are less likely to suffer damage. Flexible materials and structural systems are integral to this principle.
Image source: Internet
Damping Systems
Damping systems are a critical component of earthquake-resistant building design, and they play a pivotal role in safeguarding structures during seismic events. These systems are engineered to absorb and dissipate the energy generated by earthquakes, reducing the structural forces imposed on a building. Let’s delve deeper into the various types of damping systems and their significance:
Tuned Mass Dampers (TMDs)
Tuned Mass Dampers are one of the most widely employed damping systems in earthquake-resistant building design. These devices consist of a massive mass (the tuned mass) mounted within the structure.
The key principle behind TMDs is simple yet effective: when the building starts to sway due to seismic forces, the tuned mass moves in the opposite direction, counterbalancing the motion and reducing the overall vibration of the structure.
TMDs are particularly useful in tall skyscrapers, where the swaying motion during an earthquake can be quite pronounced. By implementing TMDs, engineers can minimize both the lateral displacement and the acceleration experienced by the building, ensuring the safety and comfort of occupants.
Viscous Dampers
Viscous dampers operate on the principle of dissipating energy through the flow of viscous fluid. These dampers are strategically placed within the building’s structure, typically at key locations such as the joints and connections. When seismic forces act upon the building, the viscous fluid within the dampers resists the motion, converting the kinetic energy into heat.
One of the advantages of viscous dampers is their adaptability. Engineers can adjust the damping characteristics by altering the properties of the viscous fluid or changing the size of the dampers. This flexibility allows for fine-tuning the damping system to suit the specific requirements of a building’s design and location.
Friction Dampers
Friction dampers, as the name suggests, rely on the frictional resistance between surfaces to dissipate seismic energy. These dampers consist of sliding or rotating plates that are attached to the building’s structure. During an earthquake, the relative motion between these plates generates frictional forces, which in turn dissipate energy.
Friction dampers are known for their simplicity and reliability. They are often used in combination with other damping systems to provide additional protection. Their low maintenance requirements make them an attractive choice for long-term building resilience.
Fluid Viscous Spring Dampers
Fluid viscous spring dampers combine the principles of fluid viscosity and spring action. These dampers consist of a piston moving within a cylinder filled with viscous fluid, connected to a spring. When seismic forces act on the building, the piston moves, compressing the spring and pushing against the viscous fluid. This action absorbs and dissipates energy.
One of the advantages of fluid viscous spring dampers is their ability to provide both stiffness (spring action) and damping (viscous action). This dual functionality allows engineers to achieve a balanced approach to seismic control, enhancing the building’s overall stability.
Damping systems are indispensable in earthquake-resistant building design.
They significantly enhance a structure’s ability to withstand seismic forces, safeguarding lives and property. The choice of damping system depends on factors such as building height, location, and structural design, with engineers carefully tailoring the system to meet specific requirements.
As technology advances, we can expect even more sophisticated damping solutions to emerge, further enhancing the resilience of our built environment in the face of earthquakes.
Materials Matter
Reinforced Concrete
Reinforced concrete is a popular choice for earthquake-resistant buildings due to its strength and flexibility. Proper reinforcement techniques are essential for its effectiveness.
Further, more importantly, the lateral load resisting system constructed with the structure is the dominant factor to safeguard against high lateral loads.
Steel Frame Construction
Steel frames offer excellent ductility and can withstand substantial lateral forces, making them ideal for seismic regions.
Image source: Internet
Cutting-Edge Technologies
Base Isolation
Base isolation is a cutting-edge seismic engineering technique that has revolutionized earthquake-resistant building design. It involves the strategic placement of flexible isolators or bearings between a building’s foundation and superstructure. The primary goal of base isolation is to decouple the building from ground motion during an earthquake, allowing it to move independently. This innovative approach offers several advantages:
How Base Isolation Works
The core principle behind base isolation is to provide a cushioning effect that absorbs and dissipates seismic energy. The base isolators, typically made of rubber and steel, serve as shock absorbers.
When seismic waves hit the building, the isolators deform and flex, isolating the superstructure from the violent ground motion.
This means that while the ground shakes during an earthquake, the building above remains relatively stable.
Benefits of Base Isolation
- Improved Building Resilience: Base isolation significantly reduces the forces transferred to the building during an earthquake. This results in less structural damage and a higher likelihood of the building remaining functional after a seismic event.
- Enhanced Occupant Safety: Buildings with base isolation systems provide a safer environment for occupants. The reduced lateral movement and sway mean less discomfort and fewer injuries during an earthquake.
- Preservation of Assets: Base-isolated structures are better equipped to protect valuable assets, such as equipment and artwork, which are less likely to be damaged or destroyed.
- Minimal Post-Earthquake Repairs: Because base-isolated buildings experience less structural damage, the cost and time required for post-earthquake repairs are significantly reduced.
- Long-Term Cost Savings: While the initial installation of base isolation systems may be more expensive, the long-term cost savings in terms of reduced maintenance and repair expenses are substantial.
Types of Base Isolators
There are several types of base isolators used in earthquake-resistant building design:
- Friction Pendulum Bearings: These isolators consist of a concave sliding surface and spherical pendulum bearings. They allow for multidirectional movement and are effective in dissipating seismic energy.
- Elastomeric Bearings: Elastomeric isolators are made of rubber and are highly effective in isolating buildings from ground motion. They are cost-effective and easy to install.
- Sliding Bearings: Sliding bearings use a sliding interface to absorb seismic forces. They are suitable for both new construction and retrofitting existing buildings.
- Hybrid Systems: Some base isolation systems combine various types of isolators to achieve optimal seismic performance. These hybrid systems are designed to address specific building requirements.
Real-World Applications
Base isolation has been successfully implemented in a variety of structures, including hospitals, museums, historical buildings, and even critical infrastructure like bridges and nuclear power plants. These applications demonstrate the versatility and effectiveness of base isolation in protecting both human lives and valuable assets.
Base isolation is a groundbreaking technology in earthquake-resistant building design. It represents a significant leap forward in mitigating the destructive impact of seismic events on our built environment.
As advancements in materials and engineering continue, we can expect base isolation systems to play an increasingly vital role in creating resilient and earthquake-resistant structures, ensuring the safety and well-being of future generations.
Seismic Bracing Systems
Seismic bracing systems are a fundamental component of earthquake-resistant building design, playing a crucial role in safeguarding structures during seismic events. These systems are designed to provide additional lateral support, effectively reducing the risk of structural damage and ensuring the safety of building occupants. Let’s delve deeper into the various types of seismic bracing systems and their significance:
How Seismic Bracing Systems Work
Seismic bracing systems consist of diagonal braces, typically made of steel, that are strategically placed within a building’s structure. These braces are designed to absorb and dissipate lateral forces generated by seismic activity. When an earthquake occurs, these braces act as shock absorbers, redirecting energy away from the building’s main structure.
Types of Seismic Bracing Systems
There are several types of seismic bracing systems used in earthquake-resistant building design, each with its unique characteristics and applications:
- X-Bracing: X-bracing, as the name suggests, forms an “X” pattern within the building’s frame. This configuration effectively resists both horizontal and diagonal seismic forces.
- K-Bracing: K-bracing resembles the letter “K” and is often used in taller buildings. It provides lateral support while allowing for vertical movement during seismic events.
- V-Bracing: V-bracing is shaped like the letter “V” and is commonly used in low- and mid-rise buildings. It offers excellent lateral stability while maintaining cost-effectiveness.
- Buckling-Restrained Braces (BRBs): BRBs are a more recent innovation in seismic bracing. These braces consist of steel tubes filled with a core material that prevents buckling under extreme loads, making them highly efficient at dissipating seismic energy.
Benefits of Seismic Bracing Systems
Seismic bracing systems offer several advantages in earthquake-resistant building design:
- Enhanced Structural Stability: By dissipating lateral forces, seismic bracing systems significantly improve a building’s structural stability during an earthquake.
- Reduction in Structural Damage: These systems minimize structural damage, leading to lower repair costs and faster post-earthquake recovery.
- Safety for Occupants: Seismic bracing systems ensure the safety of building occupants by reducing the risk of structural failure.
- Compatibility with Existing Structures: Retrofitting existing buildings with seismic bracing systems is a feasible and effective way to enhance their earthquake resistance.
Earthquake-resistant building design is a critical aspect of modern architecture. By combining scientific knowledge, innovative materials, and cutting-edge technologies, we are creating a safer world in the face of seismic threats.